NCBI Bookshelf. A service of the National Library of Medicine, National Institutes of Health.
Cappellini MD, Farmakis D, Porter J, et al., editors. 2021 Guidelines: For the Management of Transfusion Dependent Thalassaemia (TDT) [Internet]. 4th edition. Nicosia (Cyprus): Thalassaemia International Federation; 2023.
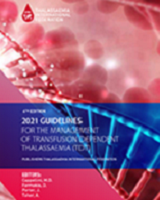
2021 Guidelines: For the Management of Transfusion Dependent Thalassaemia (TDT) [Internet]. 4th edition.
Show detailsIntroduction
Despite major advances in the treatment of thalassaemia, resulting in prolongation of the life expectancy of affected patients, a definitive cure available to all patients remained elusive until very recently. For years, the only curative treatment has been allogeneic bone marrow transplantation, limited however by its availability only to young patients with a well-matched donor (Lucarelli, Andreani and Angelucci, 2002) and the requirement for long-term immunosuppression to prevent or treat transplant-related immunological complications such as graft-vs-host-disease (GvHD) and rejection, carrying a non-negligible risk of mortality (Galanello and Origa, 2010).
Given the widespread elucidation of the genetic basis of haemoglobinopathies (Stamatoyannopoulos et al., 2001; Weatherall and Clegg, 2001), gene therapy approaches have long been envisioned by pioneers such as late Dr George Stamatoyannopoulos (Higgs, Engel and Stamatoyannopoulos, 2012). Gene therapy aspires to provide cure for thalassaemia through the manipulation of the genome of haematopoietic stem cells, thus compensating for the inadequate or faulty function of mutated genes. This can be achieved either by gene addition via a semi-random insertion of a healthy copy of the therapeutic gene into the cells using viral vectors (Dong and Rivella, 2017) or gene editing via a precisely directed mutation that repairs the gene in situ or induces a disease-modifying effect (i.e. reactivation of Hb F synthesis) using site-specific nucleases (Porteus, 2017; Antoniani et al., 2018; Boulad et al., 2018).
Ex vivo gene therapy for thalassaemia, either by gene addition or gene editing, is a haematopoietic stem cell (HSC) transplantation-based procedure. The autologous (patient-derived) HSCs are mobilised with granulocyte colony-stimulating factor (G-CSF) plus plerixafor, harvested by cytapheresis, CD34+cell-enriched by immunomagnetic separation and genetically modified ex vivo (Yannaki et al., 2012; Psatha et al., 2014; Karponi et al., 2015). The patient undergoes myeloablative conditioning followed by infusion of the gene-modified cells and remains hospitalised until the haematopoietic recovery (Finotti et al., 2015; Malik, 2016; Mansilla-Soto et al., 2016; Ferrari, Cavazzana and Mavilio, 2017; Biffi, 2018; Cavazzana and Mavilio, 2018) (Figure 1, left panel).

Figure 1
Ex vivo and in vivo gene therapy for haemoglobinopathies: the concept. G-CSF, granulocyte colony-stimulating factor; HSCs, haematopoietic stem cells; iv, intravenous; SIN-LV, self-inactivating lentiviral vectors.
Recently, in vivo approaches for gene therapy of thalassaemia, either by gene addition or gene editing, have also been preclinically pursued, aiming to overcome the current limitations of ex vivo gene therapy including the need for myeloablative conditioning, the need for transplantation expertise and high costs (Li et al., 2018; Wang et al., 2019) (Figure 1, right panel).
Gene therapy by gene addition
Gene therapy by gene addition, in other words, the transfer of a healthy copy of the β (or γ) globin gene along with its important regulatory genomic elements into target-cells, using modified viral constructs as delivery vehicles (Higgs, Engel and Stamatoyannopoulos, 2012; Mansilla-Soto et al., 2016; Cavazzana, Antoniani and Miccio, 2017), has been the most developed gene therapy approach up-to-now. After many years of preclinical evaluation, self-inactivating lentiviral vectors (SIN-LVs) have proven to be the safest and most effective means of delivery (Zufferey et al., 1997; Naldini, 2011; Naldini, Trono and Verma, 2016). In contrast to γ-retroviral vectors favouring integration in transcription start sites (TSS) and having been associated with insertional mutagenesis in gene therapy trials of X-linked severe combined immunodeficiency (SCID) (Hacein-Bey-Abina et al., 2010; Zhang, Thrasher and Gaspar, 2013) and Wiskott–Aldrich syndrome (Boztug et al., 2010; Braun et al., 2014), SIN-LVs, which preferentially integrate into actively transcribed genes rather than TSS, have not been associated with genotoxicity so far (Montini et al., 2006, 2009; Cesana et al., 2014). Extensive integration site analysis from preclinical and clinical studies involving SIN-LVs and in various disease settings, has demonstrated polyclonal haematopoietic reconstitution patterns (Aiuti et al., 2013; Biffi et al., 2013; Thompson et al., 2018; Marktel et al., 2019). Short genomic elements termed chromatin insulators have been used (Chung, Whiteley and Felsenfeld, 1993; Cavazzana-Calvo et al., 2010) or are under evaluation (Emery et al., 2000; Liu et al., 2015), concerning their ability to act as additional safety features, shielding the transgene from the influence of its surrounding genomic environment (Rivella et al., 2000).
Gene therapy by Gene editing
In gene editing approaches, site-specific nucleases − (zinc-finger nucleases (ZFN) (Carroll, 2011), transcription activator-like effector nucleases (TALEN) (Ma et al., 2013) and clustered regularly interspaced short palindromic repeats/Cas9 (CRISPR/Cas9) complex (Dever et al., 2016) are engineered to precisely identify specific genomic sequences where they create a double-strand break (DSB), which can subsequently be repaired by endogenous cellular repair mechanisms: i) in non-homologous end joining (NHEJ), a direct ligation of the two ends takes place, in an error-prone process that causes insertions or deletions (indels) leading to frameshift mutations and rendering the locus non-functional (i.e. gene knockout); ii) in homology directed repair (HDR), a donor DNA template is used and high fidelity, albeit substantially less efficient, repair of the DSB takes place, due to the resistance of haematopoietic stem cells to HDR; iii) another approach is the targeted integration of a therapeutic gene in a predetermined genomic ’safe harbour’ that supports long-term expression without interfering with the transcriptional activity of endogenous loci (Osborn et al., 2016) (Figure 2).
Genome editing eliminates the need for semi-randomly integrating viral vectors and, reasonably, the risk of insertional mutagenesis; however, the main safety concern with this approach is the unintended ‘off target’ mutagenesis that could generate additional mutations in undesired genomic loci with potential unexpected consequences.
Gene editing strategies for β thalassaemia have primarily focused on inducing reactivation of the γ globin gene (Wienert et al., 2018), aiming to correct the imbalance of the α-like/β-like globin chain ratio, the main pathophysiological cause of the disease. This can be achieved by inhibiting factors that repress the expression of the γ globin gene (i.e. BCL11A), thus mimicking hereditary persistence of fetal haemoglobin (HPFH) in which high levels of haemoglobin (Hb) F are maintained throughout adult life and compensate for the low levels of β globin expression in patients carrying thalassaemic mutations. Disrupting the binding site or the enhancer of BCL11 causes upregulation of HbF to therapeutic levels (Psatha et al., 2018). Both CRISPR/Cas9 and ZFNs are being used in ongoing gene editing clinical trials for β thalassaemia and sickle cell disease (SCD). Several other interventions at the molecular level that may provide a therapeutic result (e.g. RNA interference and forced chromatin looping) (Yannaki, Emery and Stamatoyannopoulos, 2010) are also being currently tested (Figure 2).
Gene therapy clinical trials
A. Gene addition
Bluebird bio trials
The first clinical trial phase I/II was conducted in 2007, in Paris, using myeloablative conditioning (busulfan 14 mg/kg) and a SIN-LV (HPV569, developed by Ph. Leboulch) flanked with the cHS4 insulator and encoding a β globin gene with antisickling properties (T87Q). One treated patient with compound βE/β0 thalassaemia became transfusion independent 12 months later, after a dominant clone bearing an integration site near the HMGA2 gene emerged, contributing to a third of the total haemoglobin with the rest consisting of one third of endogenous HbE and one third of HbF (Cavazzana-Calvo et al., 2010). Fortunately, this clone subsided over time without causing oncogenesis (Cavazzana, Antoniani and Miccio, 2017). Subsequently, Bluebird bio modified the vector by removing the cHS4 insulator and replacing the 5’LTR with a cytomegalovirus (CMV) promoter (vector BB305). Further clinical phase I/II studies were initiated including adults and adolescents with β thalassaemia (Northstar HGB-204), SCD (Northstar HGB-206, still ongoing) or either (Northstar HGB-205). In HGB-204 and HGB-205 trials (Mansilla-Soto et al., 2016), 15 of 22 patients with β thalassaemia became transfusion independent whereas seven had a median 73% reduction (range 19-100%) in transfusion requirements. The majority of patients (6/7) in whom best response was reduction in the number or/and the volume of transfusions belonged to the β0 genotype. In contrast, transfusion independence was achieved predominantly in patients having a non-β0/β0 genotype (12/15) over those carrying β0/β0 or 2 copies of IVSI-110 mutations (3/9). Τhe lower vector copy number (VCN) in peripheral blood as compared to the drug product VCN, indicating low engraftment of transduced cells, was strongly correlated with the lower therapeutic benefit in the β0 patients (Thompson et al., 2018). A subsequent transduction refinement was introduced in the manufacturing process of the phase III Northstar-2 HGB-207 (non-β0/β0 genotypes) and HGB-212 (β0/β0 genotypes or double IVSI-110 mutations) clinical trials, in which enrolment of 23 and 15 patients, respectively, is anticipated in USA, France, Germany, Italy, Thailand, UK and Greece. Preliminary data disclosed in June 2019, demonstrated that in HGB-207, 10/11 patients followed for ≥6 months have stopped transfusions for ≥5.9 months with a haemoglobin concentration ranging from 113 to 124 g/l (month 6 - month 18) while in HGB-212, 3/4 patients followed for ≥6 months stopped transfusions for ≥6 months having their total haemoglobin concentration ranging from 105 to 136 g/l phase I/II (Kulozik et al., 2019).
San Raffaele-TIGET
This phase I/II clinical trial was conducted in Milan and recruited 9 patients, stratified into three age groups (three adults, three adolescents, three young children). Interestingly, a myeloablative, but reduced-toxicity conditioning (treosulfan–thiotepa) was administered and the β globin SIN-LV (GLOBE)-transduced cells were infused intraosseously. Four of the total six paediatric patients became transfusion independent whereas all three adults demonstrated significant reductions (33-80%) in – but not independence from − transfusions. Integration site analysis revealed highly polyclonal haematopoietic reconstitution without emergence of clonal expansion (Marktel et al., 2019).
Other gene addition trials for thalassaemia
A phase I/II clinical trial was conducted in MSKCC, New York, in 2012 with the TNS9.3.55 β globin vector. The use of partial myeloablation (8 mg/kg busulfan) in three of four enrolled patients, provided stable engraftment but low in vivo VCN, thus resulting, at best, in reduction in transfusion requirements in one patient (Boulad et al., 2014, 2018). Those data highlighted the importance of the depth of myeloablation to obtain clinically meaningful outcomes in thalassaemia gene therapy with the currently existing globin vectors.
B. Gene editing trials
CRISPR Therapeutics uses the CRISPR/Cas9 technology for HbF reactivation in two phase I/II clinical trials for β thalassaemia (CTX001-111) and SCD (CTX001-121), recruiting 45 patients each, aged 18-35 years. A putative γ-δ intergenic HbF silencer, binding site of BCL11A, is targeted and disrupted by CRISPR/Cas9 (Antoniani et al., 2018). One β thalassaemia patient has been treated and reported transfusion-free for over 4 months post-treatment. Sangamo in another clinical trial (Thales), exploits ZFNs to disrupt the BCL11A erythroid enhancer. The study anticipates the enrolment of six patients with β thalassaemia (Holmes et al., 2018), one of whom has been reported transfusion-free for 5 weeks post-treatment with a significant increase in HbF (31%) and stable total haemoglobin levels (~90 g/l).
The long-term safety of gene therapy
Patients with hemoglobinopathies undergoing gene therapy by gene addition or gene editing need to be prospectively evaluated for at least 15 years, so as both the sustainability of clinical benefit and the long-term safety be addressed. Such patients maybe at an increased risk of developing malignancies for several reasons; first, host factors generated by the disease background or/and treatment, as in SCD, the chronic hypoxia and inflammation, clonal hematopoiesis of indeterminate potential (CHIP)-related mutations (Ghannam et al., 2020) and chronic exposure to hydroxyurea or as in thalassemia solid organ hemosiderosis, may create a permissive environment for the development of malignancies. Second, the transplant-procedure itself, either the conditioning regimen (myeloablative or non-myeloablative) or/and a low engraftment of gene-corrected HSCs allowing for clonal proliferation of the endogenous cells, constitute additional risk factors for oncogenesis. Finally, gene therapy by gene addition is associated with a low, but existing risk of insertional mutagenesis while gene therapy by gene editing has the potential to cause double strand breaks at other than the desired locations (“off-target” effects).
Up to date, in the BBB trials with the longest follow up, there have been 3 SCD patients who have developed myeloid malignancies following gene therapy by gene addition. The malignancies developed in the HGB-206 trial, at 3 and 5.5 years (MDS progressing to AML and AML, respectively) or 6 months (MDS) post gene therapy. In the first case of MDS that subsequently progressed to acute leukemia, the investigation demonstrated that the event was not gene therapy-related due to absence of vector integration in the CD34+ blast cells (Hsieh et al., 2020). In the recent case of AML, investigation has demonstrated that the vector integrated within the vesicular-associated membrane protein 4 (VAMP4) gene which has no known role in the development of malignancies whereas the patient presented several well-known genetic mutations and chromosomal abnormalities commonly observed in AML. In the early onset post-gene therapy MDS event, the diagnosis was based on trisomy 8, an MDS-related cytogenetic abnormality, but no blast cells were seen in the bone marrow and the patient is followed for the true impact of this abnormality.
Data so far suggest that in SCD patients who developed hematologic malignancies, it is unlikely the integration site of BB305 lentiviral vector to have played a role and more likely malignancies have been triggered by host factors and been exacerbated by the busulfan conditioning probably in combination with the chronic use of Hydroxyurea. There is accumulating evidence that sickle patients may be more prone to the development of myeloid malignancies (3.6 fold increase over the general population, Brunson et al., 2017), although thalassemia also, especially if transfusion-dependent, has also been associated with an increased risk of malignancies, especially hematologic ones (1.47 fold increase over the general population, Hodroj MH, Blood Reviews 2019)
Gene editing clinical studies are still in their beginning and longer follow-up in this setting also, will address the safety and the sustainability of the clinical effect.
Regulatory approval and marketing authorisation
Based on the successful clinical data from 32 adults and adolescents treated in Bluebird bio’s clinical trials, the European Commission granted conditional approval, in June 2019, for the Bluebird bio gene therapy product (autologous CD34+ cells encoding the β A-T87Q-globin gene) under the commercial name Zynteglo, to treat transfusion-dependent β+ thalassaemia in patients 12 years and older, who are eligible for stem cell transplantation but do not have a matched related donor.
The recent marketing authorization of Zynteglo and also other gene therapy products in different disease settings, with burdensome price tags has economically challenged the health system payers − even in higher income countries − who are tasked to pay a large price once, instead of paying smaller amounts repeatedly for a life-time. To encourage patient access to such highly expensive genetic treatments, new models of payment have been proposed by the stakeholders. Bluebird bio, on an outcome-based model of payment, suggested for Zynteglo five annual dose payments for patients who become transfusion-free or, as an alternative, reimbursement of only the first dose.
It remains however to be shown in the long run and on the basis of the percentage of patients becoming transfusion independent, developing a thalassaemia intermedia phenotype or failing to respond, whether indeed, such an one-time expense will be cost-effective overall, compared to the cumulative costs of life-long conventional treatment of the disease and its complications.
The current challenge of applying gene therapy
After almost 4 decades of strenuous research on haemoglobinopathies, the long-awaited gene therapy is entering the realm of clinical practice. With one gene therapy product already officially approved for β thalassaemia in Europe and more under development with promising and encouraging results in clinical trials so far, the future looks brighter than ever (Psatha, Papayanni and Yannaki, 2018). However, new challenges emerge for the thalassaemia community globally, including the costs of application of these expensive therapies on a massive scale, selection of patients clinically capable of undergoing such procedures, global access to the treatment and need for transplantation expertise to administer these sophisticated cellular treatments.
Moreover, the striking imbalance between developed and developing countries, both in terms of patient demographics (i.e. large populations of young patients in low-income countries and of adult, aged patients in high-income countries) and financial potential of health systems, makes the situation an even more complicated conundrum. Close collaboration among all stakeholders involved (healthcare professionals, patients, the pharmaceutical industry, policy makers and healthcare providers) is mandatory.

Box
Summary and Recommendations.
References
- Aiuti A., et al. Lentiviral hematopoietic stem cell gene therapy in patients with Wiskott-Aldrich syndrome. Science (New York, N.Y.). 2013;341(6148):1233151. [PMC free article: PMC4375961] [PubMed: 23845947] [CrossRef]
- Antoniani C., et al. Induction of fetal hemoglobin synthesis by CRISPR/Cas9-mediated editing of the human b-globin locus. Blood. 2018;131(17):1960–1973. [PubMed: 29519807] [CrossRef]
- Biffi A., et al. Lentiviral hematopoietic stem cell gene therapy benefits metachromatic leukodystrophy. Science. 2013;341(6148) [PubMed: 23845948] [CrossRef]
- Biffi A. Gene Therapy as a Curative Option for β-Thalassemia. New England Journal of Medicine. 2018;378(16):1551–1552. [PubMed: 29669229] [CrossRef]
- Boulad F., et al. Safe mobilization of CD34+ cells in adults with β-thalassemia and validation of effective globin gene transfer for clinical investigation. Blood. 2014;123(10):1483–1486. [PMC free article: PMC3945860] [PubMed: 24429337] [CrossRef]
- Boulad F., et al. Gene Therapy and Genome Editing. Hematology/Oncology Clinics of North America. 2018;32(2):329–342. [PubMed: 29458735] [CrossRef]
- Boztug K., et al. Stem-cell gene therapy for the Wiskott-Aldrich syndrome. The New England journal of medicine. 2010;363(20):1918–27. [PMC free article: PMC3064520] [PubMed: 21067383] [CrossRef]
- Braun C. J., et al. Gene therapy for Wiskott-Aldrich syndrome-long-term efficacy and genotoxicity. Science Translational Medicine. 2014;6(227) [PubMed: 24622513] [CrossRef]
- Brunson A., et al. Increased risk of leukemia among sickle cell disease patients in California. Blood. American Society of Hematology. 2017:1597–1599. [PMC free article: PMC5620417] [PubMed: 28830890] [CrossRef]
- Carroll D. Genome engineering with zinc-finger nucleases. Genetics. 2011;188(4):773–782. [PMC free article: PMC3176093] [PubMed: 21828278] [CrossRef]
- Cavazzana-Calvo M., et al. Transfusion independence and HMGA2 activation after gene therapy of human β-thalassaemia. Nature. 2010;467(7313):318–322. [PMC free article: PMC3355472] [PubMed: 20844535] [CrossRef]
- Cavazzana M., Antoniani C., Miccio A. Gene Therapy for β-Hemoglobinopathies. Molecular Therapy. 2017;25(5):1142–1154. [PMC free article: PMC5417842] [PubMed: 28377044] [CrossRef]
- Cavazzana M., Mavilio F. Gene Therapy for Hemoglobinopathies. Human Gene Therapy. 2018;29(10):1106–1113. [PMC free article: PMC6196755] [PubMed: 30200783] [CrossRef]
- Cesana D., et al. Uncovering and dissecting the genotoxicity of self-inactivating lentiviral vectors in vivo. Molecular Therapy. 2014;22(4):774–785. [PMC free article: PMC3982501] [PubMed: 24441399] [CrossRef]
- Chung J. H., Whiteley M., Felsenfeld G. A 5′ element of the chicken β-globin domain serves as an insulator in human erythroid cells and protects against position effect in Drosophila. Cell. 1993;74(3):505–514. [PubMed: 8348617] [CrossRef]
- Dever D. P., et al. CRISPR/Cas9 β-globin gene targeting in human haematopoietic stem cells. Nature. 2016;539(7629):384–389. [PMC free article: PMC5898607] [PubMed: 27820943] [CrossRef]
- Dong A. C., Rivella S. Advances in Experimental Medicine and Biology. Springer New York LLC; 2017. Gene addition strategies for β-thalassemia and sickle cell anemia; pp. 155–176. [PMC free article: PMC5718882] [PubMed: 29127680] [CrossRef]
- Emery D. W., et al. A chromatin insulator protects retrovirus vectors from chromosomal position effects. Proceedings of the National Academy of Sciences of the United States of America. 2000;97(16):9150–5. [PMC free article: PMC16837] [PubMed: 10908661] [CrossRef]
- Ferrari G., Cavazzana M., Mavilio F. Gene Therapy Approaches to Hemoglobinopathies. Hematology/Oncology Clinics of North America. 2017;31(5):835–852. [PubMed: 28895851] [CrossRef]
- Finotti A., et al. Recent trends in the gene therapy of β-thalassemia. Journal of Blood Medicine. 2015;6:69–85. [PMC free article: PMC4342371] [PubMed: 25737641] [CrossRef]
- Galanello R., Origa R. Beta-thalassemia. Orphanet Journal of Rare Diseases. Orphanet J Rare Dis. 2010 [PMC free article: PMC2893117] [PubMed: 20492708] [CrossRef]
- Ghannam J. Y., et al. Blood. Elsevier B.V.; 2020. Baseline TP53 mutations in adults with SCD developing myeloid malignancy following hematopoietic cell transplantation; pp. 1185–1188. [PMC free article: PMC7118814] [PubMed: 32062672] [CrossRef]
- Hacein-Bey-Abina S., et al. Efficacy of Gene Therapy for X-Linked Severe Combined Immunodeficiency. New England Journal of Medicine. 2010;363(4):355–364. [PMC free article: PMC2957288] [PubMed: 20660403] [CrossRef]
- Higgs D. R., Engel J. D., Stamatoyannopoulos G. Thalassaemia. The Lancet. 2012;379(9813):373–383. [PubMed: 21908035] [CrossRef]
- Holmes M. C., et al. A Potential Therapy for Beta-Thalassemia (ST-400) and Sickle Cell Disease (BIVV003). Biology of Blood and Marrow Transplantation. 2018;24(3):p, S172. [CrossRef]
- Hsieh M. M., et al. Myelodysplastic syndrome unrelated to lentiviral vector in a patient treated with gene therapy for sickle cell disease. Blood Advances. 2020;4(9):2058–2063. [PMC free article: PMC7218414] [PubMed: 32396618] [CrossRef]
- Karponi G., et al. Plerixafor+G-CSF-mobilized CD34+ cells represent an optimal graft source for thalassemia gene therapy. Blood. 2015;126(5):616–619. [PMC free article: PMC4520876] [PubMed: 26089395] [CrossRef]
- Kulozik A. E., et al. S140 RESULTS FROM THE PHASE 3 NORTHSTAR-3 STUDY EVALUATING LENTIGLOBIN GENE THERAPY IN PATIENTS WITH TRANSFUSION-DEPENDENT β-THALASSAEMIA AND A β0 OR IVS-I-110 MUTATION AT BOTH ALLELES OF THE HBB GENE. HemaSphere. 2019;3 S1:21–22. [CrossRef]
- Lal A., et al. Northstar-3: Interim Results from a Phase 3 Study Evaluating Lentiglobin Gene Therapy in Patients with Transfusion-Dependent β-Thalassemia and Either a β0 or IVSI-110 Mutation at Both Alleles of the HBB Gene. Blood. 2019;134 Supplement_1:815–815. [CrossRef]
- Li C., et al. Reactivation of γ-globin in adult β-YAC mice after ex vivo and in vivo hematopoietic stem cell genome editing. Blood. 2018;131(26):2915–2928. [PMC free article: PMC6024639] [PubMed: 29789357] [CrossRef]
- Liu M., et al. Genomic discovery of potent chromatin insulators for human gene therapy. Nature Biotechnology. 2015;33(2):198–203. [PubMed: 25580597] [CrossRef]
- Lucarelli G., Andreani M., Angelucci E. The cure of thalassemia by bone marrow transplantation. Blood Reviews. 2002;16(2):81–85. [PubMed: 12127951] [CrossRef]
- Ma N., et al. Transcription activator-like effector nuclease (TALEN)-mediated Gene correction in integration-free β-Thalassemia induced pluripotent stem cells. Journal of Biological Chemistry. 2013;288(48):34671–34679. [PMC free article: PMC3843079] [PubMed: 24155235] [CrossRef]
- Malik P. Gene therapy for hemoglobinopathies: Tremendous successes and remaining caveats. Molecular Therapy. 2016;24(4):668–670. [PMC free article: PMC4886952] [PubMed: 27081721] [CrossRef]
- Mansilla-Soto J., et al. Cell and Gene Therapy for the Beta-Thalassemias: Advances and Prospects. Human Gene Therapy. 2016;27(4):295–304. [PMC free article: PMC4994056] [PubMed: 27021486] [CrossRef]
- Marktel S., et al. Intrabone hematopoietic stem cell gene therapy for adult and pediatric patients affected by transfusion-dependent ß-thalassemia. Nature Medicine. 2019;25(2):234–241. [PubMed: 30664781] [CrossRef]
- Montini E., et al. Hematopoietic stem cell gene transfer in a tumor-prone mouse model uncovers low genotoxicity of lentiviral vector integration. Nature Biotechnology. 2006;24(6):687–696. [PubMed: 16732270] [CrossRef]
- Montini E., et al. The genotoxic potential of retroviral vectors is strongly modulated by vector design and integration site selection in a mouse model of HSC gene therapy. Journal of Clinical Investigation. 2009;119(4):964–975. [PMC free article: PMC2662564] [PubMed: 19307726] [CrossRef]
- Naldini L. Ex vivo gene transfer and correction for cell-based therapies. Nature Reviews Genetics. 2011;12(5):301–315. [PubMed: 21445084] [CrossRef]
- Naldini L., Trono D., Verma I. M. Lentiviral vectors, two decades later. Science. 2016;353(6304):1101–1102. [PubMed: 27609877] [CrossRef]
- Osborn M. J., et al. Gene editing and its application for hematological diseases. International Journal of Hematology. 2016;104(1):18–28. [PMC free article: PMC5595242] [PubMed: 27233509] [CrossRef]
- Porteus M. H. Advances in Experimental Medicine and Biology. Springer New York LLC; 2017. Genome Editing for the β-Hemoglobinopathies; pp. 203–217. [PubMed: 29127682] [CrossRef]
- Psatha N., et al. Superior long-term repopulating capacity of G-CSF + Plerixafor-Mobilized blood: Implications for stem cell gene therapy by studies in the Hbbth-3 mouse model. Human Gene Therapy Methods. 2014;25(6):317–327. [PMC free article: PMC4268579] [PubMed: 25333506] [CrossRef]
- Psatha N., et al. Disruption of the BCL11A Erythroid Enhancer Reactivates Fetal Hemoglobin in Erythroid Cells of Patients with β-Thalassemia Major. Molecular Therapy - Methods and Clinical Development. 2018;10(September):313–326. [PMC free article: PMC6120587] [PubMed: 30182035] [CrossRef]
- Psatha N., Papayanni P.-G., Yannaki E. A New Era for Hemoglobinopathies: More Than One Curative Option. Current Gene Therapy. 2018;17(5):364–378. [PubMed: 29357790] [CrossRef]
- Rivella S., et al. The cHS4 Insulator Increases the Probability of Retroviral Expression at Random Chromosomal Integration Sites. Journal of Virology. 2000;74(10):4679–4687. [PMC free article: PMC111989] [PubMed: 10775605] [CrossRef]
- Stamatoyannopoulos G., et al. The Molecular Basis of Blood Diseases. 3rd edn. Philadelphia: W.B. Saunders; 2001.
- Thompson A. A., et al. Gene Therapy in Patients with Transfusion-Dependent β-Thalassemia. New England Journal of Medicine. 2018;378(16):1479–1493. [PubMed: 29669226] [CrossRef]
- Wang H., et al. In vivo hematopoietic stem cell gene therapy ameliorates murine thalassemia intermedia. The Journal of clinical investigation. 2019;129(2):598–615. [PMC free article: PMC6355219] [PubMed: 30422819] [CrossRef]
- Weatherall D. J., Clegg J. B. The Thalassaemia Syndromes, The Thalassaemia Syndromes: Fourth Edition. In: Weatherall D. J., Clegg J. B., editors. Oxford, UK: Blackwell Science Ltd; 2001. [CrossRef]
- Wienert B., et al. Trends in Genetics. Elsevier Ltd; 2018. Wake-up Sleepy Gene: Reactivating Fetal Globin for β-Hemoglobinopathies; pp. 927–940. [PubMed: 30287096] [CrossRef]
- Yannaki E., et al. Hematopoietic stem cell mobilization for gene therapy of adult patients with severe Β-thalassemia results of clinical trials using G-CSF or plerixafor in splenectomized and nonsplenectomized subjects. Molecular Therapy. 2012;20(1):230–238. [PMC free article: PMC3255592] [PubMed: 21952171] [CrossRef]
- Yannaki E., Emery D. W., Stamatoyannopoulos G. Gene therapy for β-thalassaemia: The continuing challenge. Expert Reviews in Molecular Medicine. 2010;12(October):1–20. [PubMed: 20883576] [CrossRef]
- Zhang L., Thrasher A. J., Gaspar H. B. Current progress on gene therapy for primary immunodeficiencies. Gene Therapy. Gene Ther. 2013:963–969. [PubMed: 23719067] [CrossRef]
- Zufferey R., et al. Multiply attenuated lentiviral vector achieves efficient gene delivery in vivo. Nature Biotechnology. 1997;15(9):871–875. [PubMed: 9306402] [CrossRef]
- Hematopoietic stem cell transplantation for people with β-thalassaemia.[Cochrane Database Syst Rev. 2021]Hematopoietic stem cell transplantation for people with β-thalassaemia.Sharma A, Jagannath VA, Puri L. Cochrane Database Syst Rev. 2021 Apr 21; 4(4):CD008708. Epub 2021 Apr 21.
- Growth hormone therapy for people with thalassaemia.[Cochrane Database Syst Rev. 2020]Growth hormone therapy for people with thalassaemia.Ngim CF, Lai NM, Hong JY, Tan SL, Ramadas A, Muthukumarasamy P, Thong MK. Cochrane Database Syst Rev. 2020 May 28; 5(5):CD012284. Epub 2020 May 28.
- Review Growth hormone therapy for people with thalassaemia.[Cochrane Database Syst Rev. 2017]Review Growth hormone therapy for people with thalassaemia.Ngim CF, Lai NM, Hong JY, Tan SL, Ramadas A, Muthukumarasamy P, Thong MK. Cochrane Database Syst Rev. 2017 Sep 18; 9(9):CD012284. Epub 2017 Sep 18.
- A genetic combination of silent beta-thalassaemia, high Hb A2 beta-thalassaemia, and single alpha globin gene deletion causing mild thalassaemia intermedia.[J Med Genet. 1984]A genetic combination of silent beta-thalassaemia, high Hb A2 beta-thalassaemia, and single alpha globin gene deletion causing mild thalassaemia intermedia.Galanello R, Maccioni L, Rosatelli MC, Ibba P, Nurchi AM, Cao A. J Med Genet. 1984 Apr; 21(2):153-6.
- Review When to consider transfusion therapy for patients with non-transfusion-dependent thalassaemia.[Vox Sang. 2015]Review When to consider transfusion therapy for patients with non-transfusion-dependent thalassaemia.Taher AT, Radwan A, Viprakasit V. Vox Sang. 2015 Jan; 108(1):1-10. Epub 2014 Oct 7.
- Gene therapy for thalassaemia - 2021 GuidelinesGene therapy for thalassaemia - 2021 Guidelines
- Nucleotide Links for Gene (Select 54609) (9)Nucleotide
Your browsing activity is empty.
Activity recording is turned off.
See more...